Environment & Energy
Related: About this forumThermodynamics of Electrochemical Marine Inorganic (Carbonate, CO2 (aq.)) Carbon Removal
The paper I'll discuss in this post is this one: Thermodynamics of Electrochemical Marine Inorganic Carbon Removal Fabian J. Dickhardt, Michael P. Nitzsche, Simon Rufer, T. Alan Hatton, and Kripa K. Varanasi Environmental Science & Technology 2024 58 (52), 22953-22966.
In 2023, after the expenditure of trillions of dollars on so called "renewable energy," the world reportedly released 37.78 billion tons of carbon dioxide in part by consuming dangerous fossil fuels, led by dangerous coal (which people keep saying is "dead" although it is no such thing) at 15.4 billion tons, dangerous petroleum, 12.2 billion tons, and dangerous natural gas, 7.4 billion tons. The balance comes from cement manufacture, flaring and "other" categories.
Breakdown of Carbon Emissions by Source; 2003-2023
In addition, land use changes, which presumably includes land destroyed stupidly for so called "renewable energy" related rendering of wilderness into industrial parks for so called "renewable energy" junk - wind and solar industrial parks - that will last from less than 2 decades to a maximum of 3 decades before be coming landfill, added about 3.6 billion tons of CO2 to the atmosphere. The total mass of "new" carbon dioxide added each year is thus 41.4 billion tons.
It is said, that like claims about batteries, and worse, hydrogen, like claims about carbon capture and sequestration, that efforts to remove carbon dioxide from the atmosphere are futile and represent nothing more than attempts to greenwash fossil fuels. There is, I concede, some merit in this argument, although even in these most dire times, with the fall of the United States not merely imminent but underway in an act of national suicide, I personally am willing to believe that the subject is one worthy of consideration and research. I think this true if one feels that the proposal for a closed carbon cycle, as proposed by the late Nobel Laureate George Olah, has merit.
(Olah was one of the Martians (Hungarian immigrant scientists) who emigrated to the United States in past times, when the United States was a beacon of at least the hope of decency, a democracy invested in science and the arts and higher aspirations, including those of sustainability, rather than the racist, sexist apartheid oligarchic hell into which it degenerated finally in 2025.)
Olah made this proposal in 2011 six before his death in 2017:
Anthropogenic Chemical Carbon Cycle for a Sustainable Future George A. Olah, G. K. Surya Prakash, and Alain Goeppert Journal of the American Chemical Society 2011 133 (33), 12881-12898.
The problem with closing the carbon cycle is this: It takes energy, massive amounts of energy, and of course, to avoid being a perpetual motion machine of a material (and thermodynamic) type, the energy in question must be carbon free, sustainable, and - this I think is critical - reliable and continuously available.
Anyone who knows anything of my thinking will recognize that I consider only one form of primary energy that qualifies, nuclear energy, period.
The paper cited at the outset of this post makes an estimate of how much energy would be required just to remove carbon dioxide from the environment from the environmental matrix in which it is most volumetrically concentrated, seawater. It comes out of MIT, one of the universities that still has a nuclear engineering program, nuclear engineering programs being the last, best, hope of the human race.
From the paper's introduction:
Synopsis
This work details a comprehensive framework to assess the energetics of electrochemical marine carbon dioxide removal (mCDR) systems and their impacts on marine chemistry.
Introduction
It is widely accepted among the scientific and international communities that anthropogenic emissions of carbon dioxide, methane, and other greenhouse gases are causing a warming of the atmosphere and impacting the global climate. (1) Mitigation of the most severe consequences of climate change will require wide-scale decarbonization at all levels of our society through strategies including energy efficiency measures, (2) electrification with renewable or nuclear energy sources, (3) and abatement of emissions through carbon capture, utilization and sequestration (CCUS). (4,5) Beyond mitigation or abatement of greenhouse gas pollution at the source, processes to capture and sequester environmental greenhouse gases at the gigaton scale are considered crucial to achieving net-zero or net-negative emissions. (6−8)
While development of environmental carbon removal technologies has largely focused on processes for direct air capture (9−11) and bioenergy with carbon capture and sequestration (BECCS), (12,13) the oceans have also come into focus as a reservoir for CO2 removal as its large interface with the atmosphere and capacity for tens of teratons of CO2 are responsible for the absorption of approximately 30% of current anthropogenic CO2 emissions. (14) While this offsets atmospheric warming potential, the associated acidification results in harm to calcifying marine life. (15) Ocean-based technologies for carbon dioxide removal (CDR) aim to increase the oceans carbon dioxide uptake while mitigating ocean acidification by leveraging the higher volumetric concentrations (approximately 100 mg/L) and alternate chemical pathways available in the ocean relative to the atmosphere. (16) Further, by utilizing the natural interface between ocean and atmosphere for CO2 uptake, mCDR relies entirely on the ocean as natural sorbent, promising high environmental compatibility if implemented correctly.
Marine CDR (mCDR) technologies can be broadly divided into biotic and abiotic processes. Biotic mCDR processes involve sequestration of atmospheric CO2 through primary production of organic or inorganic carbon. Processes for acceleration of natural carbon cycles include seeding with iron or other nutrients, (17) farming of aquatic plants, promoting ecosystem recovery, (18) and artificial up- and downwelling. (19,20) Abiotic mCDR processes drive CO2 drawdown through the pH-dependence of dissolved inorganic carbon through alkalinity enhancement or direct removal via pH modulation. (20) While all techniques ultimately shift the carbonate equilibria in seawater, chemical technologies for carbon removal and sequestration primarily focus on directly adjusting alkalinity to shift dissolved inorganic carbon (DIC) to aqueous CO2 for degassing (21−27) or to carbonate ions to facilitate (1) direct removal via calcium carbonate precipitation (22,28−31) or (2) alkalinity enhancement driven carbon absorption by the ocean. (25,30,32−34) The involved carbonate species (CO2(aq), HCO3- and CO32-) are termed dissolved inorganic carbon (DIC) with speciation dictated by the following equilibria:
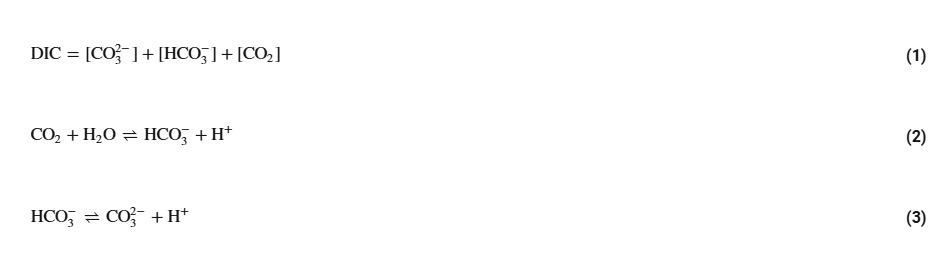
The process described herein is an electrochemical process, and despite much nonsensical hoopla connected with the unsupportable - to the point of being delusional - that electricity is "green," it is no such thing. Electricity is a thermodynamically degraded form of energy, meaning that it destroys the inherent exergy of dangerous fossil fuels. (The charging of batteries or the hydrolysis of water to make hydrogen further degrades the exergy of the dangerous fossil fuels overwhelmingly used to generate electricity.)
Other processes are conceivable, for instance the use of very high temperatures. In supercritical water, the solubility of carbon dioxide is actually much higher than in subcritical water, but in the subcritical (hot) state, much lower than at room temperature, which offers some means of separation of the gas from water.
(cf. Gerasymchuk, Y., Wędzyńska, A. & Stręk, W. Liquid Syngas Based on Supercritical Water and Graphite Oxide/TiO2 Composite as Catalyst for CO2 to Organic Conversion. Catal Lett 152, 28402851 (2022)
I have made a highly speculative argument, speculative in the sense that the psychological barriers, including the will to sustain the environment, but also an economic argument based on the moral position that no one seems willing to provide for future generations, that California might provide all of its urban and agricultural needs, and its need for environmental water, such as might be required for the restoration of Owens Lake, which was drained to feed Los Angeles, by supercritical water desalination:
The Energy Required to Supply California's Water with Zero Discharge Supercritical Desalination.
A "back of the envelope" (actually Excel) calculation suggests that processing the roughly 130 billion cubic meters of water consumed and flowing in natural systems would involve processing water containing about 13 billion tons of carbon dioxide, roughly 30% of the annual dumping rate of the gas in our fossil fueled world.
However, the paper at hand refers to electrochemical means which may or may not superior.
Returning to the paper then goes on to discuss the acid/base chemistry of the ocean which it discusses as "total alkalinity" drawing in the borate and sulfate buffering systems that control the (falling) pH of the ocean.
It's illustrative to look at some schematic graphics of the processes examined in the paper:
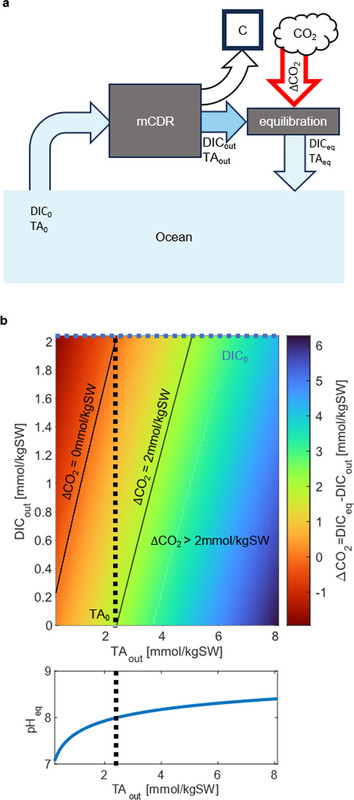
The caption:
(Greek letters have been substituted with word equivalents to address the inability of the DU editor to accommodate these.)
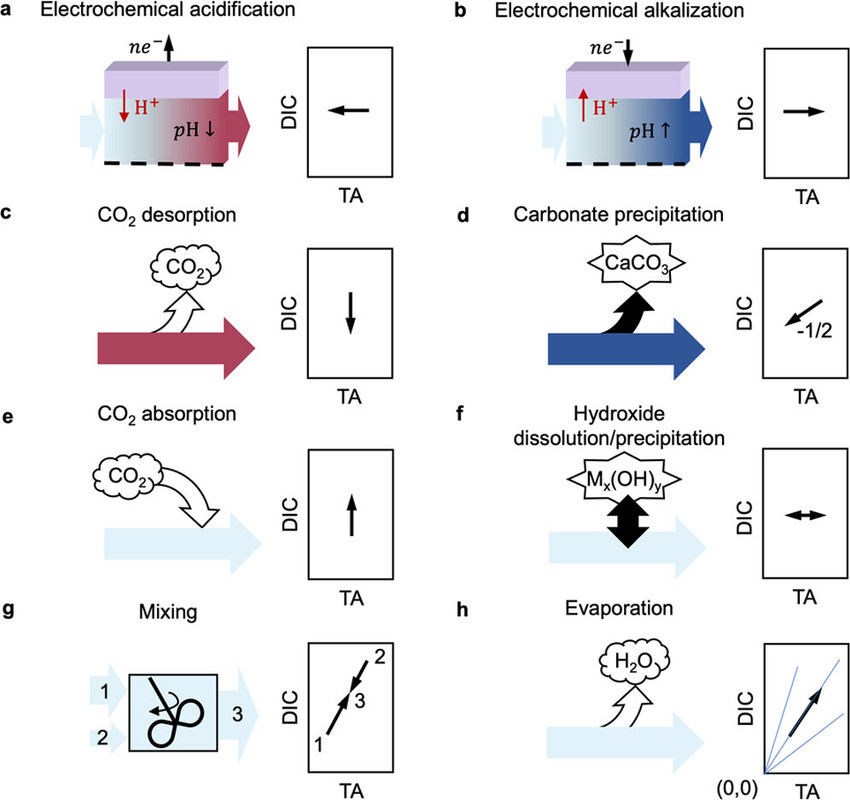
The caption:
DIC = dissolved organic carbon. TA=total alkalinity.
The electrochemical thermodynamics are governed by a famous equation known as the Nerst equation, which governs electrochemical cells, the most common type being batteries, although many other types of electrochemical cells exist.
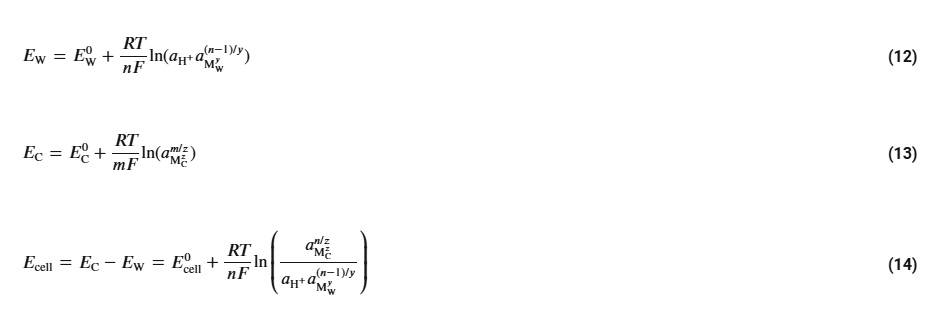
The subscripts in these equations refer to mediating species, cations and anions other than carbonate. The superscripts refer to ionic charges of these species.
In seawater, the expanded deviation from ideality, is given by the following version of the Nernst equation:
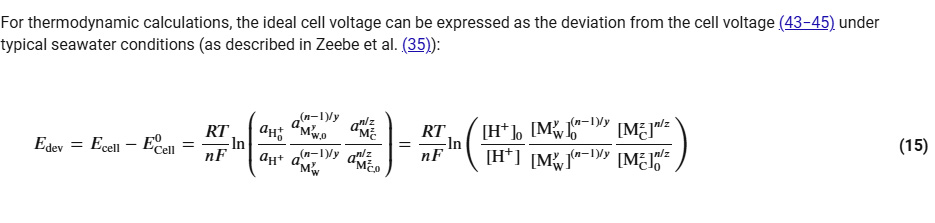
In thermodynamics, energy flows depend on the path used in any energy consuming or generating system, where the path greatly affects the recoverable (useful) work (exergy) and the losses - which are inevitable 100% of the time - to entropy.
For this reason, thermodynamic calculations often appeal to a class of integrals known as "line integrals" or "path integrals" usually designated by circle in the integration symbol as in equation 16 in the paper:

The equation above is simplified further in the paper to delineate the fact that the mediating ions behave in a symmetric fashion.
A number of schematics of different approaches to the problem are presented in the paper, suggesting different paths, in particular alkali swing systems. (Dr. Heather Willauer at the US Naval Laboratories has written about the use of ion selective membranes to make jet fuel out of seawater using electrical power.) CO2 is more readily absorbed into water in basic systems, particularly those at low temperatures.
There are a number of schematics of different approaches to these systems, including a step in some where the inorganic carbon is produced by precipitation of calcium carbonate by the addition of lime. These represent alkalinity swing systems. The captions of these various approaches are elaborately detailed in the captions of the diagrams
To save time, and to overcome the limitations of DU graphics, I will only show one of the 4 such diagrams in the paper, this as a graphic object:
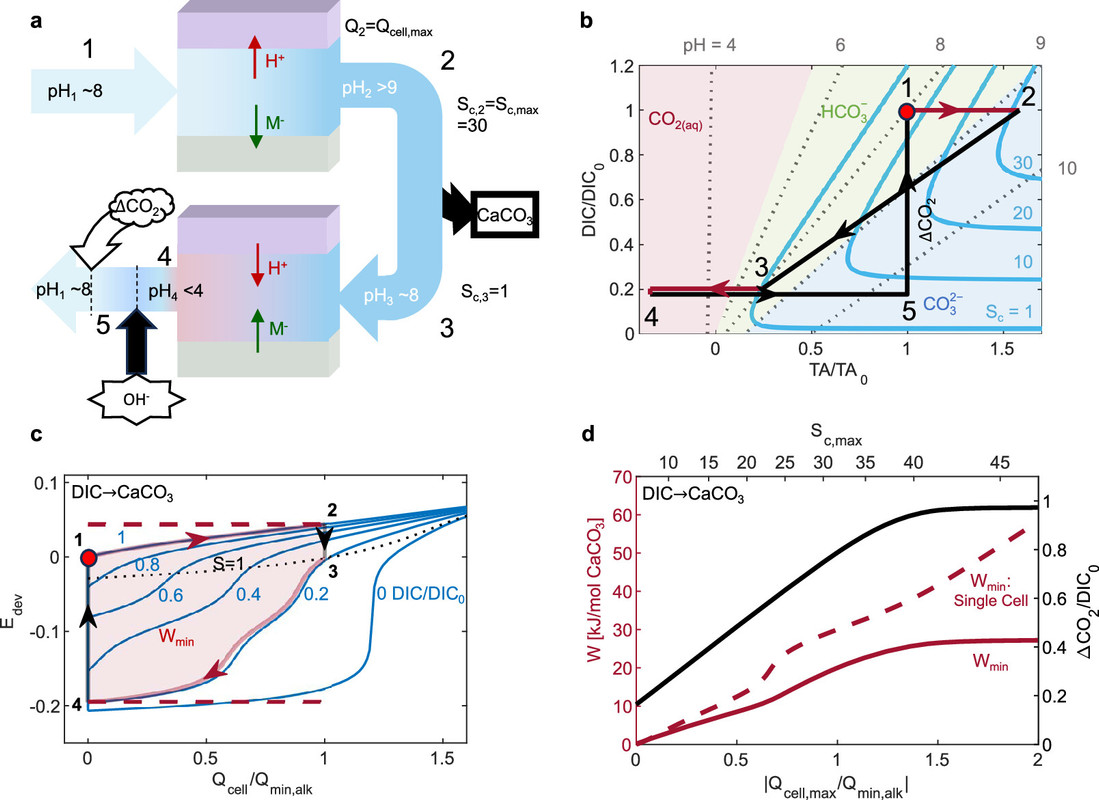
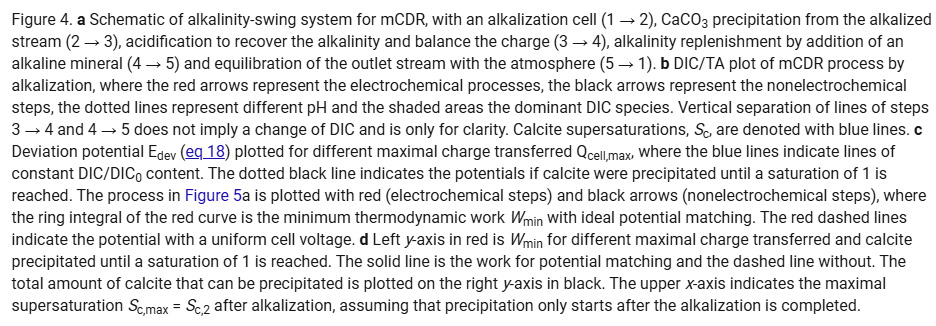
The important concluding graphics show the energy requirements for the isolation of carbon dioxide from seawater. Note that this is only the isolation cost, and has nothing to say about what to do with the CO2 after isolation, although as I suggested above, it could be used in a closed carbon cycle where the convenient fluid fuels, the best of which would be dimethyl ether, and structural polymers are manufactured (at an additional energy cost) directly from CO2 rather than dangerous fossil fuels.
To appreciate these graphics a note about a "bypass ratio" as described in the paper is relevant:
The next two graphics rely on this distinction:
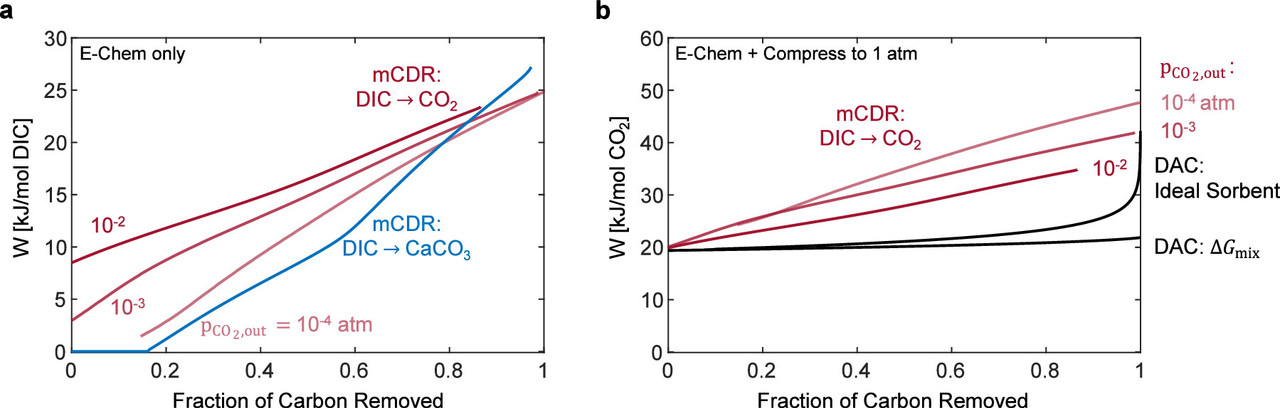
The caption:
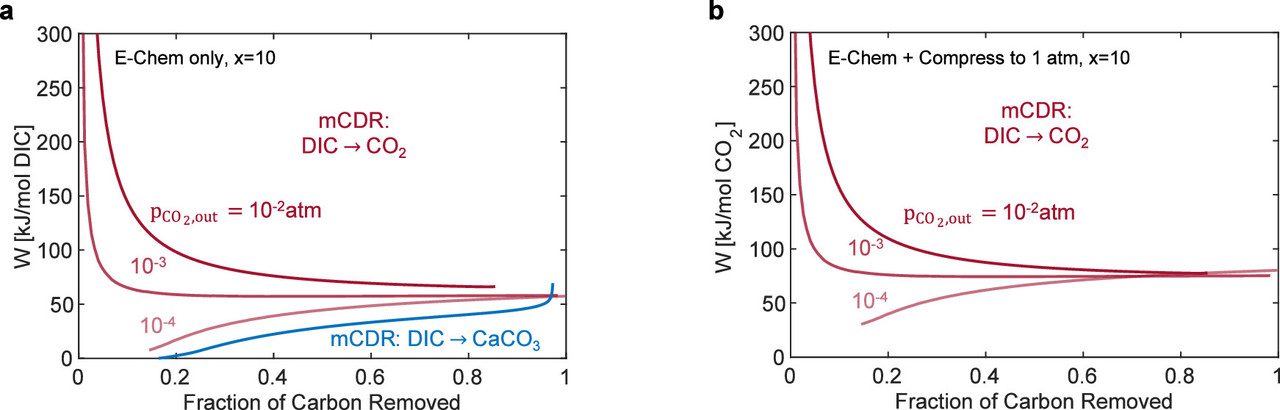
The caption:
The lowest energy cost of isolation only that is reasonable (as it includes the cost of compression) is figure 7b, which visually suggests an energy cost of around 45 kJ/mol for the removal of dissolved inorganic carbon from seawater. The molecular weight of carbon dioxide is 44.0095 grams/mol, meaning a per gram isolation energy cost of roughly 1.02 kJ/gram. A metric ton of CO2 thus requires 1.02 billion Joules of energy to isolate from seawater. It follows that the energy cost of removal of one year's worth of carbon dioxide released to the atmosphere in 2023 amounts to 4.43 X 1019 Joules of energy, 44.3 Exajoules.
This is, happily, an amount of energy that is accessible, since world energy demand is much greater than this, 642 EJ as shown in the following table from the 2024 World Energy Outlook published by the IEA.
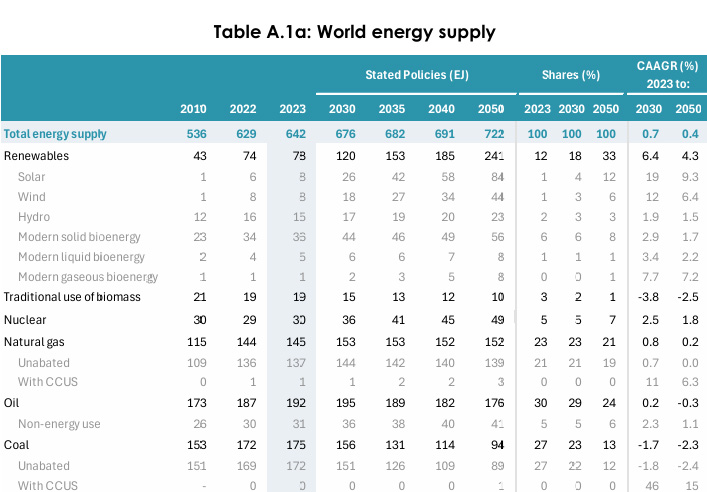
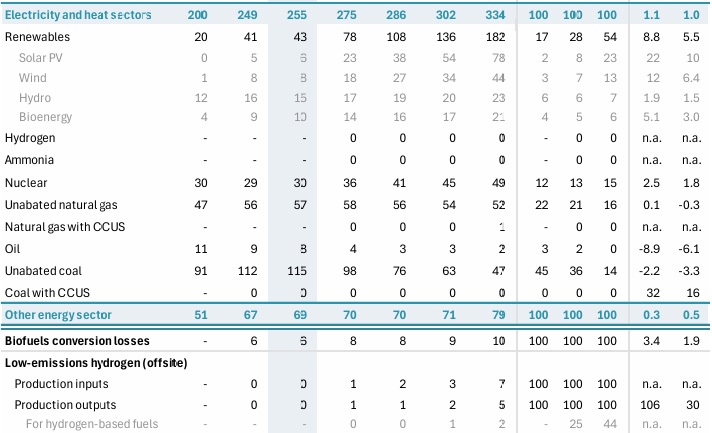
IEA World Energy Outlook 2024
Table A.1a: World energy supply Page 296.
In a climate of vituperation based on appeals to fear and ignorance, the world supply of the only infinitely expandable form of climate change energy, nuclear energy, still managed to produce in 2023 around 30 EJ of primary energy as heat. Because of the industrial superiority of China, this figure is likely to once again begin to grow, albeit not here. A typical nuclear reactor in the existing fleet is a Rankine cycle device with a thermodynamic efficiency of roughly 33%, since they are overwhelmingly used to generate electricity with small amounts being sent for district heating in some areas. A few have moderately higher thermal efficiencies.
I have argued in this space and elsewhere that via process intensification, the recovery of thermal energy as exergy might approach numbers greater than 70%, although this might include the capture of chemical energy in the form of hydrogen which would be available for captive use, along the lines outlined by George Olah, the Martian, discussed above, to make methanol or DME, the wonder fuel dimethyl ether.
During my weekend technical exchange with my son, who is seeking a Ph.D. in nuclear engineering focusing on nuclear materials science, we discussed the Olah scheme along with some technical issues he's come across in refractory materials (which are not his direct research focus but of which he is aware from other members of his group.). In this conversation, I set out the task of combinatorial optimization to address corrosion in the decomposition of sulfuric acid at high temperatures. (I have promised to send him this paper, along with some electronic textbooks I have on electrochemistry, on which he is working.)
We live in the age of the triumph of ignorance, such that the happy thoughts of feasibility this paper evokes must be counterbalanced by the fact that fear and ignorance are ascendent.
In issues of energy, as much as I hate to say it, I must acknowledge that on our end of the political spectrum we have not been particularly wise. We've cheered insipidly for trashing huge stretches of wilderness for the fossil fuel dependent solar and wind scam, which has done nothing to address the collapse of the planetary atmosphere other than to accelerate it. Experiment supersedes theory, and the theory that we could save our asses by a reactionary return to the middle ages when our energy demands could be addressed almost solely by appeal to the weather, weather we've destabilized by our actions, has experimentally failed, and done so miserably, tragically indeed.
I will escape life soon enough myself, but the kernel of these ideas may survive, if not in me, then perhaps with my son and his colleagues, and surely, I have hope, elsewhere perhaps with the authors of this paper.
Perhaps humanity may yet rise phoenix-like from the ashes of its ignorance.
Have a nice evening.